Photoconductive Detectors
Definition: photodetectors which exhibit a reduction in electrical resistance caused by incoming light
Alternative terms: photoresistors, light-dependent resistors, photocells
More general term: photodetectors
Opposite term: photoemissive detectors
German: Photowiderstände
Categories: light detection and characterization, optoelectronics
How to cite the article; suggest additional literature
Author: Dr. Rüdiger Paschotta
Photoconductive detectors are a type of photodetectors which are based on photoconductive semiconductor materials. Here, the absorption of incident light creates non-equilibrium electrical carriers, and that reduces the electrical resistance across two electrodes. There are also some exotic cases with negative photo conductivity, i.e., with an increase of resistance caused by illumination.
Alternative terms for photoconductive detectors are photoresistors, light-dependent resistors and photocells.
While for the detection of visible light one would usually prefer photodiodes due to their clearly superior performance (except sometimes in cost-critical applications), photoconductive detectors are often used as infrared detectors.
In principle, one could consider photodiodes to be photoconductors, at least when used in photoconductive mode, i.e., with a negative bias voltage. However, in this article we assume photoconductors to be devices without a p–n junction. The electrical characteristics are profoundly different from those of photodiodes; for example, one obtains a substantial dependence of the photocurrent on the applied bias voltage.
Photoconductive antennas are often used to detect terahertz radiation. Although such devices can also be called photoconductive detectors, in this article we consider only detectors for light, i.e., electromagnetic radiation with much higher optical frequencies.
Intrinsic and Extrinsic Photoconductors
An important distinction is that between intrinsic and extrinsic photoconductors:
Intrinsic Materials
In an intrinsic material, one exploits interband transitions, i.e., optical transitions across the band gap from the valence band to the conduction band. This is possible only when the photon energy is above the band gap energy, i.e., for a sufficiently short optical wavelengths.
For example, lead salt, cadmium sulfide and mercury cadmium selenide detectors are based on that operation principle.
Extrinsic Materials
In an extrinsic material for impurity band detectors, one exploits additional energy levels introduced by impurities. These are introduced by doping with additional chemical species and provide additional excitation pathways. High doping concentrations are preferable to obtain strong absorption, so that rather thin layers can be used; only, for too high doping the dark current and noise properties are degraded.
For allowing particularly high doping concentrations, blocked impurity band (BIB) detectors have been developed, which contain an additional intrinsic layer, called the blocking layer, besides the heavily doped active layer. The intrinsic layer has the function to suppress hopping carriers in the impurity band.
Examples for extrinsic materials are silicon and germanium, doped with arsenic, copper, gold, or indium. They are mostly used for detecting infrared light with particularly long wavelength, and are dominant in that applications beyond 20 μm.
Device Design
A photoconductive detector can simply consist of a piece of semiconductor material with two attached metallic electrodes for sensing the resistance. Instead of simply attaching two electrodes to opposite sides of a semiconductor chip, one will typically apply serpentine-shaped electrodes to the surface of a semiconductor chip and illuminate the area between them. Light hitting the electrodes is then lost, if the illumination comes from the side of the electrodes. However, it is also possible to use back illumination, i.e., having the electrodes on the side opposite to the one where the light enters the semiconductor. Generally, one tries to keep the electrode distance small (for reasons which become apparent below), and for a large active area one consequently needs serpentine-like electrode structures.
Due to the typically strong light absorption, a rather thin semiconductor layer (e.g. with a thickness of only a few micrometers) is sufficient. It can be a polycrystalline layer which is deposited with a simple evaporation technique.
In contrast to photodiodes, no p–n junction is required, so that the device design of photoresistors is simpler. It is also not necessary to apply epitaxial growth of highly pure semiconductor material; the fabrication of a polycrystalline layer is normally sufficient. Therefore, photoconductive detectors can be realized with materials which are hardly suitable for the fabrication of photodiodes. In particular, that includes materials which are suitable for detection of infrared light at relatively long optical wavelengths; see the article on infrared detectors.
Problem of Thermal Excitation
Particularly for photoconductors requiring only small excitation energies, as required for detecting long-wave infrared light, additional carriers can be generated by thermal excitation, and the resulting signals can of course mask real signals from incident light. It is not only that one obtains an additional constant current, but also the corresponding noise.
That problem is often mitigated by operation at reduced temperatures. In some cases, a moderate reduction of temperature with a Peltier (thermoelectric) cooler is sufficient – and sometimes primarily used to eliminate the effects of temperature variations –, while other devices are operated at much reduced temperatures as such as 100 K or in extreme cases even 4 K. In some cases, not only the detector chip, but also an infrared filter needs to be cooled, because otherwise thermal radiation from the filter may produce unwanted signals in the detector.
A problem which can not be solved by cooling the detector or filter is background thermal radiation coming from objects near the observed objects. This is a problem, for example, when detecting a weak infrared signal coming from the sky, together with infrared radiation from the atmosphere.
Responsivity and Bandwidth
The responsivity of a photoconductive detector is proportional to the mean lifetime of the generated carriers: the longer such a carrier lives, the longer it can contribute to the photocurrent. On the other hand, the photocurrent is inversely proportional to the transit time of the carriers: the faster a carrier can get through the device structure, the more it contributes to the current (which means charge per unit time). With a quite simplified physical model, one can show that the responsivity (ratio of photocurrent to incident optical power) is given by
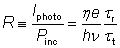
where the first term is the same as for a photodiode and the ratio τr / τt of the recombination time and the transit time is called the photoconductive gain Gp, which depends on the applied voltage U and the carrier mobility μ:
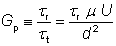
where d is the electrode distance. The photoconductive gain can be substantially larger than unity, which means that the obtained photocurrent for a given light power (i.e., the responsivity) can be much larger than that of a photodiode. This is possible only because minority carriers reaching an electrode can lead to the injection of additional majority carriers; otherwise, an excited carrier could contribute to the photocurrent for no longer than its transit time.
A high responsivity can be achieved by using a photoconductive material with long carrier recombination time and higher carrier mobility. Further, the electrode distance should be small, since a larger distance not only increases the distance over which carriers must travel, but also decreases the internal electric field.
Unfortunately, a long carrier relaxation time, as is useful for obtaining a high responsivity, also leads to a small detection bandwidth. Nevertheless, one often uses materials with quite long carrier relaxation times.
The response of photoconductive detectors is often quite nonlinear – very much in contrast to that of photodiodes. This is essentially due to the role of the carrier lifetime, which is limited by the recombination rate, that depends on the carrier density. For quantifying that nonlinearity, some manufacturers specify a γ value based on resistance values measured for two different values of the illuminance, e.g 10 lux and 100 lux; they define γ = lg(R10 lux / R100 lux, which would be unity in case of a linear response. Cadmium selenide photoresistors, for example, can have γ values around 0.6 to 0.8.
Dark Resistance
It is desirable that a photoconductive detector has a high dark resistance, i.e., exhibits only a low electrical conductivity without incident light. The dark resistance can be of the order of 1018 Ω or even higher for some detectors (e.g. high-quality CdS detectors), while it is far lower for others (e.g. low-quality CdS). Tentatively, it is lower for long-wavelength detectors.
The term dark current is not common for photoconductive detectors; it would depend on the applied bias voltage.
Noise
Different types of noise can affect the output signal of a photoconductive detector:
- There is a thermal noise, also called Johnson noise, arising from the thermal movement of carriers. Its strength depends on the resistance and the operation temperature.
- There is noise arising from generation and recombination of carriers.
- In addition, there is some amount of 1/f noise, which hurts mostly at low frequencies.
There can of course be additional noise from a preamplifier, which however is often negligible for high quality amplifiers.
Common Photoconductors
The following semiconductor materials are mostly used:
Cadmium-based Detectors
Cadmium sulfide (CdS) detectors mostly respond to light in the blue-green spectral region. They have been widely used in various consumer devices such as cameras (as light meters), automatic light control devices and as detectors in light barriers, mostly because they are fairly cheap. However, their use is increasingly restricted e.g. through the RoHS ban on cadmium; they can often easily be replaced with other devices, e.g. silicon photodiodes.
The response times of cadmium sulfide detectors are in the millisecond regime, e.g. between 5 and 100 ms. in addition, there are pronounced memory effects, i.e., the response significantly depends on the illumination history, even over time scales of seconds. Such phenomena can be explained with physical models involving different types of recombination centers and trapping centers, introduced by crystal defects.
In some cases, the slow response of CdS has been exploited in electronic circuits, e.g. for dynamic loudness compressors.
Higher-quality cadmium sulfide detectors can be fabricated, where a thin CdS film is deposited on a sapphire substrate. With such devices, it is possible to obtain very high dark resistance and detect optical powers on the picowatt level.
Similar detectors can be made with cadmium selenide (CdSe), which has its peak spectral response at longer wavelengths (around 0.7 μm). One can also use the ternary compound CdSxSe1−x with intermediate properties.
Lead Salts
Lead sulfide (PbS) has been recognized as a photoconductive materials for infrared light early on, and is suitable for wavelengths up to ≈3 μm. Lead tellurite (PbTe) is a similar material, but with a narrower bandgap and thus allows operation up to ≈7 μm. Both materials have been developed mainly for military applications (e.g. heat-seeking missiles) during the second world war and thereafter. Later on, they have largely been replaced with mercury cadmium telluride (see below).
Mercury Cadmium Telluride
Mercury cadmium telluride (MCT, HgxCd1−xTe, but somewhat non-stoichiometric) is an intrinsic material which can provide good performance even at relatively long infrared wavelengths beyond 10 μm. Through the composition parameter x, one can tune the band gap energy in a wide range from 0.8 μm (CdTe) to essentially zero, because HgTe is a semimetal (having no bandgap). MCT with a suitable composition can be used in the atmospheric windows at 3–5 μm and 8–12 μm. The latter wavelength range is quite suitable for thermal imaging even at low temperatures and with high precision.
MCT detectors are usually cooled during operation, mostly below 200 K or even below 100 K – but less than some other detector materials for similarly long wavelengths (e.g. extrinsic silicon). A Stirling cooler is typically used. The required operation temperature may be reached within a few minutes after turn-on.
Mercury cadmium telluride is of course a rather poisonous and therefore problematic material. Unfortunately, it is difficult to replace in some applications. Therefore, they are some temporary exceptions e.g. within the European RoHS regulations, allowing the use of that material for some applications.
For some common applications like the thermal imaging of buildings, one would normally not use MCT, but e.g. devices based on microbolometers, although they cannot reach the same performance levels.
Extrinsic Silicon and Germanium
Silicon is not only widely used as an intrinsic material for photodiodes, but also in the form of an extrinsic material for long-wave infrared detection. In the latter case, it is heavily doped with arsenic, copper, gold, or indium. It is then suitable for very long wavelengths, partly even beyond 200 μm wavelength. It needs to be cooled to very low temperatures (e.g. 4 K with liquid helium), because thermal excitation would otherwise mask any infrared light signals.
An interesting aspect is that extrinsic silicon detectors can be made with CMOS and CCD technology, and can thus be well used for infrared image sensors. In that case, they are usually operated as charge-transfer devices, operating essentially like conventional CCD chips. They are also realized in the form of focal plane arrays. However, the required very low operation temperature partially leads to preferences for mercury cadmium telluride, except for the very long wavelength region.
Germanium can similarly be used as an extrinsic material and has some advantages for the very long wavelengths. However, it is less popular than silicon – partly because of its much lower solubility for impurities, and also because silicon technology is generally much better developed.
Suppliers
The RP Photonics Buyer's Guide contains 11 suppliers for photoconductive detectors.
Questions and Comments from Users
Here you can submit questions and comments. As far as they get accepted by the author, they will appear above this paragraph together with the author’s answer. The author will decide on acceptance based on certain criteria. Essentially, the issue must be of sufficiently broad interest.
Please do not enter personal data here; we would otherwise delete it soon. (See also our privacy declaration.) If you wish to receive personal feedback or consultancy from the author, please contact him e.g. via e-mail.
By submitting the information, you give your consent to the potential publication of your inputs on our website according to our rules. (If you later retract your consent, we will delete those inputs.) As your inputs are first reviewed by the author, they may be published with some delay.
See also: photodetectors, infrared detectors, photoconductive sampling, photoconductive switches
and other articles in the categories light detection and characterization, optoelectronics
![]() |
If you like this page, please share the link with your friends and colleagues, e.g. via social media:
These sharing buttons are implemented in a privacy-friendly way!